Lecture 17 - Stellar Death (3/18/99)
Stellar Evolution --- | ---
White Dwarfs/Neutron Stars
Reading:
Chapter 17-1, 17-2 (ZG4)
Notes:
pages 68-72
Strange rings around the remnant of SN1987A as seen by HST.
(Courtesy
STSCI)
 |
Key Question: |
What is a supernova?
|
 |
Key Principle: |
Gravitational Potential Energy
|
 |
Key Problem: |
To calclate the energy and luminosity
of a supernova
|
Investigations:
- White Dwarfs
- What are white dwarf stars?
- What are they composed of?
- Why do they sometimes have hydrogen lines?
- How to they acquire magnetic fields up to 10^4 T?
- What is the surface gravity of a solar mass white dwarf?
- What is the escape velocity from the surface of a white dwarf?
- What fraction of the rest mass energy is liberated by accretion
onto a white dwarf?
- How does the radius of a white dwarf scale with mass?
- Why is there a maximum mass for a white dwarf?
- What happens if a white dwarf accretes sufficient mass to put it
over the Chandrasekhar limit?
- Supernovae
- What is a supernova?
- What is the difference between a Type I supernova and a
Type II supernova?
- Why is the core collapse in a massive star forming a Type II supernova
so rapid?
- What role does photodisintegration play during the iron core
collapse?
- How many neutrinos are produced in a supernova and why?
- How many neutrinos were detected on Earth from the Supernova 1987a
(SN1987a) which was seen in the Large Magellanic Cloud (LMC)?
- What role does the reverse shock wave from the core collapse play
in a Type II supernova?
- Why does the light curve from a supernova show the
characteristics of the radioactive decay of Cobalt and Nickel?
- What is the characteristic Luminosity of a Type II supernova and
how do we estimate it?
- Why might Type I supernovae form in a binary star system and how?
- What is mass transfer in a binary system?
- What are the Lagrange points in a two-body system and how are
they related to the Roche lobes of a binary system?
- Why are Type I supernovae more luminous than Type II supernovae?
Supernovae and Supernova Remnants
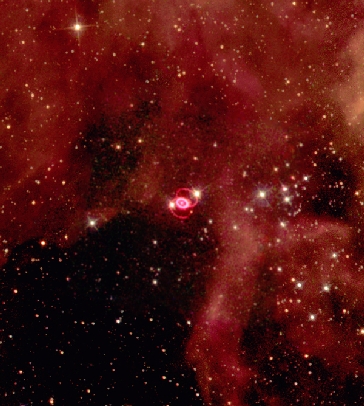
Remnant of Supernova 1987a, as seen in the
Hubble Heritage picture, courtesy HST/STSCI
There are a number of spectacular images of supernove, supernova remnants,
and other stellar graveyards. Some examples:
Stellar Remnants in Outline:
- Massive Stars
- For massives stars, M > 3 solar masses, the carbon - nitrogen -
oxygen core formed after helium fusion ends will become hot
enough for carbon fusion to commence upon contraction.
- For masses 3 to 9 Msun, this core is degenerate when fusion
begins, and a runaway ignition occurs called carbon
detonation, analogous to the helium flash.
- Carbon detonation is more violent than the helium flash (hence
its name) and may well destroy the star. It is likely, however,
that the star manages to survive carbon detonation relatively
unharmed.
- Stars more massive than about 9 solar masses will have a
non-degenerate C-N-O
- After carbon fusion begins, the star once again makes a loop
in the H-R diagram with core carbon fusion followed by collapse
of the inert core and carbon shell burning.
- The more massive of these stars are massive enough to pass
through several stages of fusion of heavier and heavier elements
at ever increasing densities and temperatures.
- The behavior of these stars will be similar to the helium burning
medium mass stars with mass loss and ejection of the envelope into
planetary nebulae.
- However, two things will disrupt this process: the formation of an
iron core, and increase of core density beyond the electron degeneracy
limit of 1.4 Msun.
- When an inert iron core is formed after fusing carbon to oxygen -
magnesium - neon, and neon - oxygen to silicon, and silicon to iron,
you can no longer extract energy from fusion to heavier nuclei.
The binding energy of the iron nucleus (per nucleon) is a minimum,
and heavier elements are less tightly bound, so it would actually
use up energy to fuse iron into a heavier element. Likewise, breaking
apart iron into lighter fragments would also use up energy. Iron
is the end of the line for thermonuclear energy generation!
- In addition, as the iron core contracts in these stars generating
gravitational energy and high temperatures, it passes the electron
degeneracy limit of 1.4 Msun. The degeneracy cannot withstand the
increasing core gravity and collapses to ever smaller radii, increasing
in density and temperature.
- At this point two things happen: the iron nuclei in the core begin to
be broken apart by the high-energy photons (gamma rays) from the
extremely high blackbody temperatures generated in the contracting
core, and the electrons are squeezed into the nuclei themselves
removing their pressure support from the core.
- When the wavelength of the electrons becomes small enough that they merge with the protons in the iron nuclei, they are captured and
the two particles become neutrons (this is basically the same
reaction as the start of the proton-proton chain, with the outgoing
positron replaced with an incoming electron):
p + e -> n + v
The neutrino v carries away the energy of this reaction, as
it can stream straight out of the core of the star without being
absorbed (it requires the weak interaction, which means that it is
unlikely to happen). This effectively uses up energy from the core
since the neutrinos escape with it, like little tiny thieves.
- The fragmentation of the iron from the gamma ray photons into
silicon and magnesium (for example) uses up energy also, supplied
by the photons.
- The result of these two processes is that energy is removed from the
core, removing pressure support, causing it to contract further,
increasing these reactions which in turn remove more energy. This
is another runaway catastrophic process due to positive feedback.
- Within a fraction of a second, the core collapses entirely releasing
a flood of neutrinos. There is effectively no pressure support, and
the collapse happens at free-fall. With the tremendous gravitational
forces of the super-dense core, speeds of 70,000 km/s
(about 0.25c)
are achieved by the outermost layers of the core (note the core is
only a few thousand kilometers in size at this point).
- The iron core electrons and protons are quicky converted into neutrons.
Thus the entire core is now made of neutrons.
- The density in this neutron core rise during the collapse until
the point of neutron degeneracy is reached. This is equivalent
to electron degeneracy, but occurs at smaller distances because the
neutron is about 2000 times more massive than the electron.
- When the neutron degeneracy limit is reached, the core collapse
is suddenly halted as the neutron degeneracy pressure takes over.
- For the collapsing core, this is like hitting a brick wall. The outer
parts of the core are collapsing at 70,000 km/s and run into the
forming degenerate core, causing a "bounce" or rebound. This
in turn runs into the material following the infalling core also
at high velocity, causing a shock wave to propagate outward
through the star.
- This shock wave, like that caused by a supersonic airplane, or
a large explosion, or an earthquake, carries tremendous energy,
and as it blasts out through the stars envelope it blows the
star completely apart. It is a vast tidal wave carrying all before
it, hurling the material from around the core (rich in heavy
elements) outward at speeds of around 10,000 km/s.
- This titanic explosion (caused by implosion of the core) is called
a supernova.
- Supernovae
- Supernovae release tremendous amounts of energy, and for a short
while outshine everything else. At maximum brightness, supernove
emit visible light with a luminosity of 10^10 Lsun! (Q:
What absolute visual magnitude does this correspond to?)
- The light emitted by the supernova is only a tiny fraction of
the total energy (less than 0.001 of the total). The "luminosity"
of the neutrinos produced in the first second is about 10^46 Watts!
- Supernova suddenly appear a bright new stars in the sky. They
grow in brightness until they reach a maximum luminosity, then
as the explosion subsides they fade away over the course of a
couple years.
- Supernovae have been recorded throughout history. Bright new stars
were obviously cause for some excitement, even though they
would then disappear.
- Arab astronomers reported a new star in 1006 AD.
- Chinese astronomers reported a "guest star" in 1054 AD
(as well as the 1006 supernova and onein 1181).
- Tycho Brahe reported a new star in 1572 and Johannes Kepler
one in 1604. The fact that new stars could appear in the sky
help overturn the idea of the immutable heavens.
- These were all visible with the naked eye and
occured in our own galaxy.
- Calculations show that we should expect a supernova somewhere in
or very near our Milky Way galaxy every 25 to 100 years, though
many of these will be hidden behind dust clouds or on the far
side of the galaxy.
- There has not been a galactic supernova seen for nearly 400 years.
However, in 1987, a bright supernova in a small neighbor galaxy
gave modern astronomers their first glimpse of a supernova in
action.
- In addition to seeing supernovae themselves, we can also study
the disrupted star-stuff they leave behind: a supernova
remnant.
- In the center of the supernova remnant will be the leftover
core of the star, now compacted down to neutron degenerate
densities comparable to that of the atomic nucleus itself.
This is a neutron star.
- The neutron star will initally be very hot from the collapse
(billions of degrees K), but tiny in size. It will therfore
be very faint because of its tiny surface area (remember
L=R^2T^4) and hard to see.
- The Crab Nebula
- Supernova remnants tend to be shell-like nebulae, not to be
confused with planetary nebulae. There are shell-like
remnants from Tycho's and Kepler's supernovae, and the
supernova of 1006 (SN1006).
- These shells are the blasted off envelopes of the star.
Q: At a speed of 10,000 km/s, how far would the shell
of SN1006 be from the central star now?
- However, not all supernova remnants look like this.
- In 1781, the French astronomer Charles Messier compiled a catalogue
of celestial objects likely to be confused with comets. These
included nebulae, star clusters, and other such objects. This
catalogue is known as the Messier catalogue and the objects in
it, the "Messier" objects, are often targets of telescopic
observations even today.
- The first object in Messier's catalogue, designated M1, is in
the constellation Taurus, and appears to be a diffuse nebula.
It is also known as the "Crab Nebula" for its vague crablike
shape.
- The "guest star" reported by the Chinese in 1054 AD. was also
in the constellation Taurus, in the location now occupied by
the Crab nebula.
- Astronomical measurements show that the Crab Nebula is about
1.35 pc in radius, and is expanding at a velocity of 1400 km/sec.
At this expansion rate, the Crab Nebula was created about 900
years ago, consistent with its being the remnant of SN1054.
- However, the Crab Nebula does not appear like most other supernova
remnants. It is not a shell, or fragments of shells. It appears
to be a filled volume, called a plerion. It also has
numerous filaments and tendrils.
- The Crab Nebula is also relatively bright, emitting a considerable
amount of radiation from radio waves up to X-rays.
- The luminosity of the Crab Nebula is about 3 x 10^31 J/s (Watts),
or about 10^5 Lsun! Where does this energy come from?
- It could be left over energy from the 10^44 Joules liberated
in the supernova, though it appears that there is something
in the nebula actively supplying the power.
- Supernova 1987A
- A supernova was discovered in the southern sky on February 24, 1987.
- It was located in the Large Magellanic Cloud (LMC), about 53,000
parsecs from the Sun. Light takes about 160,000 years to get here
from there.
- The official designation of the supernova was SN1987A, the first
(A) supernova (SN) discovered in 1987.
- At discovery, the apparent visual magnitude of SN1987A was around 5,
over the following weeks it brigtened to about 3rd magnitude.
- At the point of discovery, SN1987A was about 20 hours "old", that
is since the core collapse itself (plus the 160,000 years for the
light to get here!).
- Progenitor star was about 20 solar masses (spectral type O) when
on the main sequence, formed around 10 million years ago, with main
sequence luminosity of about 60000 Lsun.
- Just before the explosion, it was a blue supergiant, on the horizontal
branch (after becoming a red giant, then supergiant) with a luminosity
of 10^5 Lsun. In the core it was burning silicon, oxygen, neon,
carbon, helium, and hydrogen in concentric shells about the forming
iron core.
- At the time of core collapse, the core reached a temperature
of about 200 billion K and a collapse velocity of 70,000 km/s.
The collapse took a fraction of a second.
- The total luminosity liberated was around 10^44 Joules. For
about a month, SN1987 put out a luminosity of over 10^8 Suns.
- Almost all of the energy of the supernova came out in light
weakly-interacting neutrinos. About 10^58 neutrinos were produced
in the core collapse. On Feb 24, 1987, about 10^13 neutrinos
passed through your body from the supernova! About a million people
on the Earth had an "interaction" with a neutrino, of course with
no noticable effect.
- During this period, there were several neutrino detection experiments
running on the Earth. These were designed to measure neutrinos coming
from nuclear fusion in the Sun's core. Two of these experiments,
one in Japan, and one in a mine near Cleveland under Lake Erie,
detected neutrinos from the supernova!
- Astrophysicists at Penn were involved in the Japanese Kamiokande
experiment.
- These experiments detected about a dozen neutrinos each from SN1987A.
They used tons of water as a detector with phototubes to detect the
light emitted by particles produced after the interaction.
Since the supernova was in the southern sky, the neutrinos detected
had already passed through the Earth!
- These precious neutrinos confirmed the theories of the core collapse
trigger for supernovae. Furthermore, the energy of the neutrinos gave
the core temperature at collapse - about 2x10^11 K!
- SN1987A is still being monitored to study the earliest stages of
supernova remnant formation and to look for the appearance of
a pulsar in the remnant.
- What's Left Over
- If the core mass is less than 1.4 Msun, we are left with a white
dwarf, supported against gravity by electron degeneracy.
- A star up to 7 - 8 Msun can lose enough mass through winds and
planetary nebula formation to be left with a 1.4 Msun core.
Stars from 8 to 10 Msun may be able to also form a O-Mg-Ne
white dwarf.
- If it has a mass greater than this, then the core left over has a
mass greater than 1.4 Msun, and it undergoes core collapse in
a supernova explosion.
- The remnant is supported by neutron degeneracy, as the electrons were
captured on the protons and converted to neutrons (emitting copious
neutrinos). This is called a neutron star.
- The 1.4 Msun limit for a white dwarf versus a neutron star is called
the Chandrasekhar limit after the astrophysicist who first
calculated it.
- A neutron star has over 1.4 Msun of mass compressed to densities
of 10^11 kg per cm^3! The radius of a neutron star is about
10-15 km (the size of downtown Philadelphia).
Prev Lecture ---
Next Lecture ---
Astr12 Index ---
Astr12 Home
smyers@nrao.edu
Steven T. Myers